
8.2. Building Integrated Solar Energy Technologies
Solar energy conversion is a large topic. The key technologies to mention here include:
- Photovoltaics (PV, optoelectronic systems) - convert solar visible radiation into electricity;
- Concentrating Solar Power (CSP, solar thermal, or optocaloric systems) - convert solar thermal radiation into electricity;
- Solar heating systems - utilize solar heat (concentrated or not) without conversion.
You can learn these technologies in depth, taking some of the courses in the Solar Energy Option of RESS. Here in this lesson, we will turn to one of the application examples of PV technology to study the factors that affect the practical implementation of solar panels in buildings. But before doing that, let us review the basic principles by which photovoltaic systems operate.
PV Basics
Photovoltaic (PV) technology is one of the ways to convert solar resource into usable energy - specifically sunlight to electricity. Photovoltaic conversion is enabled by certain semiconductor materials, which have a property to generate electric current when they absorb incident photons. The physics of the photovoltaic effect can be schematically envisioned as three step process [Brownson, 2014]:
- absorption of light;
- generation of charge carriers (electrons and holes);
- separation of charge carriers (so that they can perform work).
This effect is physically realized in certain semiconductor materials - for example, monocrystalline silicon, polycrystalline silicon, amorphous silicon, cadmium telluride, and copper indium gallium selenide/sulfide and some others, all of which can be potentially used for making the PV cells.
Watch This Video as an introduction to PV cell technology and the photovoltaic effect.
This first short video (2 min) provides an animated illustration of the photovoltaic effect:
Video: Energy 101: Solar PV (2:00)
All right, we all know that the sun's energy creates heat and light. But it can also be converted to make electricity-- and lots of it. One technology is called Solar Photovoltaics, or PV for short. You've probably seen PV panels around for years, but recent advancements have greatly improved their efficiency and electrical output. Enough energy from the sun hits the Earth every hour to power the planet for an entire year. Here's how it works.
You see, sunlight is made up of tiny packets of energy called photons. These photons radiate out from the sun and about 93 million miles later, they collide with a semiconductor on a solar panel here on earth. It all happens at the speed of light. Take a closer look and you can see the panel is made up of several individual cells, each with a positive and a negative layer, which create an electric field. It works something like a battery. So, the photons strike the cell, and their energy frees some electrons in the semiconductor material. The electrons create an electric current, which is harnessed by wires connected to the positive and negative sides of the cell. The electricity created is multiplied by the number of cells in each panel and the number of panels in each solar array.
Combined, a solar array can make a lot of electricity for your home or business. This rooftop solar array powers this home. And the array on top of this warehouse creates enough electricity for about 1,000 homes. OK, there are some obvious advantages to solar PV technology. It produces clean energy. It has no emissions, no moving parts. It doesn't make any noise, and it doesn't need water or fossil fuels to produce power. And it can be located right where the power is needed, in the middle of nowhere. Or it can be tied into the power grid. Solar PV is growing fast, and it can play a big role in America's clean energy economy, anywhere the sun shines.
Next, let us gather some more details on the structure, functions, parameters of the photovoltaic materials.
Reading Assignment:
The PVEducation website is very good articulating many technical aspects of photovoltaic systems. Although we do not intend to study the PV physics here in detail, definitely bookmark this website as a useful resource.
Spend no more than 1 hour browsing through this website and self-learn or refresh the PV technology background.
PV Application in Buildings
Current applications of solar photovoltaics are adapted to the following scales:
- portable (small electronics - e.g., calculators, computers, etc.);
- distributed power generation (homes, isolated equipment);
- utility scale power (solar farms).
In the scale-up process, the single PV cells are combined to modules, and modules can be arranged into arrays:
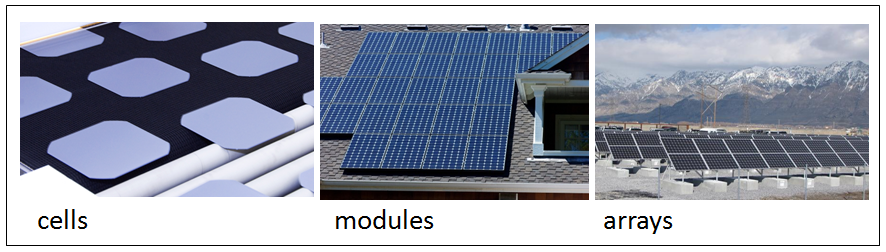
In this lesson, we choose one of the applications – the building integrated photovoltaics (BIPV) – for more detailed consideration as an interesting example of sustainable technology implementation.
The building-integrated photovoltaics (BIPV) are multifunctional materials that are both structure-supporting and power-generating at the same time. There are some advantages and challenges associated with this technology, which are summarized in table below:
Advantages | Challenges |
---|---|
Lower cost due to multi-functional nature of materials and due to more efficient design. Currently, PV contributes 0.5% to national energy demand; DOE expects it to reach 50% (!) with BIPV implemented. | System orientation, weatherability, durability are the factors that affect the system performance. Those parameters may be not easy to optimize if it is BIPV rather than add-on PV. |
Improved aesthetics: panels are produced as facade lining, roof tiles, slates, shingles, windows (glazing, etc.). | BIPV modules can be heavier than regular building elements. Because of potential overload, new building standards and codes need to be in places to regulate this integration. |
Thin-film PV can be applied directly to conventional building materials because the flexible and light. | BIPV modules are costly compared to regular add-on PV due to their multifunctional value, but that is not always recognized. BIPV is not mass-produced, but rather custom made, and that adds to the price. |
Social acceptance: not many see long-term value because PV technology is thought to be soon outdated. There should be more acceptance from contractors and end-users alike. |
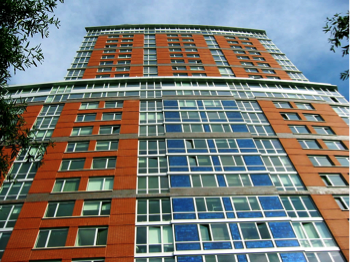
While the main function of a conventional PV system is efficient power generation, the building integrated PV systems, which become the components of the building envelope must satisfy a number of additional requirements, such as:
- appearance: color, image, size;
- weather-tightness;
- wind loading;
- durability and maintenance;
- safety during construction and in use (fire, electrical, structure stability);
- cost.
Reading Assignment:
Report: Building Integrated Photovoltaics: An Emerging Market, Greentech Media Inc., 2010.
This review summarizes factors affecting the market of the BIPV technology, related policies, and its promise.
In order to address all the necessary factors, the PV integration should be discussed early in the beginning of the design process. There are following PV integration options [Robert and Guariento, 2009]:
(a) Shading systems
These include louvers, either horizontal or vertical, which may be mounted outside the building over windows or balconies. Their function is to shade the windows from excessive light. The main issue with these structures is to make them resistant to wind load and easily accessible for maintenance. These subscreens can be made adjustable to maximize the sunlight gain.
(b) Rainscreen systems
Cladding panels can be used to protect the load-bearing external walls from rain water (especially masonry and concrete). PV panels can perform the function of such cladding and tiles. These structures are usually vertical. The ventilated cavity between the exterior cladding and the main wall help keep down the operating temperature of PV cells, enhancing their performance and provide space for cables. PV rain screens have moderate power output due to vertical orientation.
(c) Stick-system curtain walls
Curtain walls are used in those buildings that have internal columns or structural steel to support the main loads. Curtain walls are not weight-bearing and their main function is to resist air and moisture infiltration; also, building insulation is often attached to them (warm facade). A popular solution is to build the curtain walls from aluminum and steel framing filled with glass panels. PV panels can be sealed into curtain wall structures in both vision area or opaque area of the facade instead of regular glass.
(d) Unitized curtain walls
The unitized wall segments are pre-assembled at a factory and then are delivered to the building site. The controlled industrial environment provides more precision and quality for sealing the PV panels into the wall framework and making cable connections.
(e) Double-skin facades
Glass facades are often designed as "double-skin", i.e., when there is significant air space between the internal and external glass walls. This helps to reduce heat transfer losses through the walls. PV modules can be readily integrated into the external facade. Ventilation through the double-skin structure provides valuable cooling on the back of the panels.
(f) Atria and canopies
For the highest performance, PV are best integrated into horizontal or tilted elements, such as atria and canopies. These structures are usually free from overshadowing and are easy to ventilate. However, these structures may be more prone to heat transfer losses compared to plain opaque or insulated.
Supplemental Reading on BIPV technology:
Book: Robert, S. and Guariento, N., Building Integrated Photovoltaics. A Handbook, Birkhauser Verlag AG, 2009.
This handbook on BIPV technology (available online through PSU library) provides many details that are beyond the capacity of this course. If you have specific interest in this area of technology, you may find this book a useful resource.
NREL Report: Eiffert, P., Kiss, G.J., Building-Integrated Photovoltaic Designs for Commercial and Institutional Structures" NREL, 2000
This report contains multiple examples of BIPV design, which complement the description of this technology.
Lifecycle Considerations
One of the main reasons why solar PV systems are being developed is their potential to replace fossil fuel combustion in power generation. While economically, fossil fuels are proved to be profitable, there is a sustainability concern (coal, oil, and gas will run out eventually) and there is an environmental concern (combustion pollutes and contributes to the greenhouse effect on earth). The solar PV can potentially address both of these concerns.
Because, unlike fossil fuels, solar energy is virtually unlimited, PV seems to be a perfect solution from the standpoint of energy sustainability. (Sunlight will not run out any time soon).
From the standpoint of environmental protection, the main question is whether or not the environmental benefit of reducing CO2 emissions achieved through the use of the solar systems will offset the impact of possible toxic emissions from the manufacturing of the solar modules and potential land pollution from the disposal of those systems at the end of their lifetime.
Let us make some rough estimations of the environmental benefit of PV technology. Consider the following example.
Example
Question: How much CO2 emissions will be mitigated by employing PV for electric power generation?
The European Photovoltaic Industry Association predicted that by 2030 the implemented PV units will be able to produce 2,646 TWh of electricity annually worldwide. This roughly accounts for 9% of the total population's needs.
Imagine this amount of energy to be produced by burning coal instead:
The general reaction of carbon combustion is: C(coal) + O2 = CO2
We know that the average coal energy content is ~24 MJ/kg.
We also know that the average efficiency of coal-fired power plants is ~33%, so, roughly, we need three times as much energy generated by coal combustion for obtaining the specified amount of electric energy:
Energy by combustion = Target electric energy / 0.33
To unify the units, convert the global PV energy to joules: 2,646 TWh = 9.53 × 1018 J
So, the energy we would need to generate through coal combustion is:
9.53 × 1018 J / 0.33 = 2.89 × 1019 J
Now, we can find how much coal we need to generate this amount of energy by dividing the amount of energy by coal energy content:
Amount of coal needed = 2.89 × 1019 J / 24 × 106 J = 1.2 × 1012 kg (=1200 million ton)
Now, based on the assumed 95% content of carbon in the coal (let us consider anthracite coal), we calculate the amount of pure carbon burnt in the process:
1.2 × 1012 × 0.95 = 1.14 × 1012 kg C
Because for each mole of carbon in the coal, there is 1 mole of CO2 produced, we can find the amount of CO2, taking into account the molar masses of the components – C 12 g/mol and CO2 44 g/mol, as follows:
Amount of CO2 released = 1.14 × 1012 kg × (44/12) = 4.18 × 1012 kg CO2 (= 4180 Tg CO2)
This quite substantial mass of CO2 will not be released to the atmosphere due to the operation of the PV systems around the world. Let us compare this number to the total global CO2 emissions, currently estimated at 31,350 Tg CO2 [IEA Statistics, 2012]
4,180 / 31,350 × 100% = 13.3%
This is the fraction of CO2 emissions that would be prevented due to employment of PV technology (if it is employed as predicted by the European Photovoltaic Industry Association).
We should also remember that PV is only one of the promising renewable technologies on the list. All of those technologies are locale-sensitive and therefore will complement each other in global power generation. Localization of technologies by resource would also help reduce energy expenditures and emissions from transportation.
The above example demonstrates the scale of the benefit. Now, let us look at the other side of the medal. The accelerated growth of PV system manufacturing and use will have to become a major market and also should have infrastructure for maintenance and disposal of vast amount of PV-related components. While PV panels are considered are relatively durable with a long projected service life, high volume annual turnover of such systems can be expected. Here, we can do another estimation exercise.
Example
Questions:
- How many solar panels would be needed in the fossil fuel free economy?
- How many panels would need to be replaced and disposed off each year?
Precise analysis is hardly possible, since we cannot predict the market trends and know for sure which renewable technologies will be major in the distant future. So, we just create a hypothetical case with a few assumptions:
Assumption 1: There are no more fossil fuels left, so all energy has to come from renewable sources.
Assumption 2: Global energy demand is split evenly among the six major technologies: solar PV, solar thermal, wind, geothermal, hydro-power, and biomass. This is probably unrealistic, but let us take it as a starting point for simplicity. In this case, PV power generation has to cover 1/6 of the total energy demand.
Assumption 3: The total global energy demand is 13 TW of power (this is currently estimated value, which we will use in this example): 13 TW → 4.1 × 1020 J (per year)
Then, the fraction of energy that has to come from PV is:
1/6 × 4.1 × 1020 = 6.833 × 1019 J (per year)
Let us consider 250 W silicon monocrystalline panels (64 x 39 x 1.57 in size; 44 lb (20 kg) in weight) as the main functional unit for this estimation.
If the panels are installed at a favorable location, we can take the measured output of 1200 kWh per kW of capacity per year. This is a typical output factor for the locations with favorable insolation [Vanek and Albright, 2008]. To explain this a little better: the nominal capacity of the solar system (250 W in this case) indicates the system output at full insolation; but the system does not operate at full insolation all the time (due to varying sun position and seasons). So, instead of the theoretically possible maximum output of 2190 kWh, the system produces only 1200 kWh per year. This value can be empirically estimated and is termed “regional factor”.
Then, we can find how much energy this panel will produce per year:
1200 (kWh/kW) x 0.25 kW = 300 kWh (= 1.08 × 109 J)
Finally, we divide the total energy demand by the unit panel output to find # panels:
Number of panels required = 6.833 × 1019 J / 1.08 × 109 J = 6.327 × 1010
63 billion panels (!) is many, but if we divide this number by current living population (~ 7.7 billion), we get 8.2 panels per person, which seems quite reasonable.
To answer the second question, let us make the following assumption:
Assumption 4: The panel lifetime is assumed to be 50 years.
In this case, 1/50 of all panels will need to be replaced each year:
63/50 = 1.26 billion panels
If all this decommissioned equipment is simply disposed off, the total volume of waste generated per year will range up to 8.1 × 107 m3. If this waste in deposited in 20 m high dump, it would cover the area of 4.05 km2. (That means, the dump will cover the area of my home town in 9 years.)
The above-illustrated issue of waste disposal associated with the proliferation of the current PV technology calls for further development of solar cell design. The following improvements will be needed:
- development of thin, low volume solar elements;
- increasing efficiency of energy conversion to minimize the size of systems;
- designing cells for recyclable turn-over and conservation of valuable materials;
- using low-toxicity chemicals and materials in manufacturing;
- creating infrastructure for lifecycle management.