
Global sustainability system
To set the framework for applying sustainability principles to engineering activities and technologies, let us for a while widen the angle of our view and first look at the global interconnections. Later on, when zooming in to particular processes and technologies, some of these global elements and loops may remain beyond the boundaries of our viewfinder. However, it will be important to keep these large-scale connections in mind.
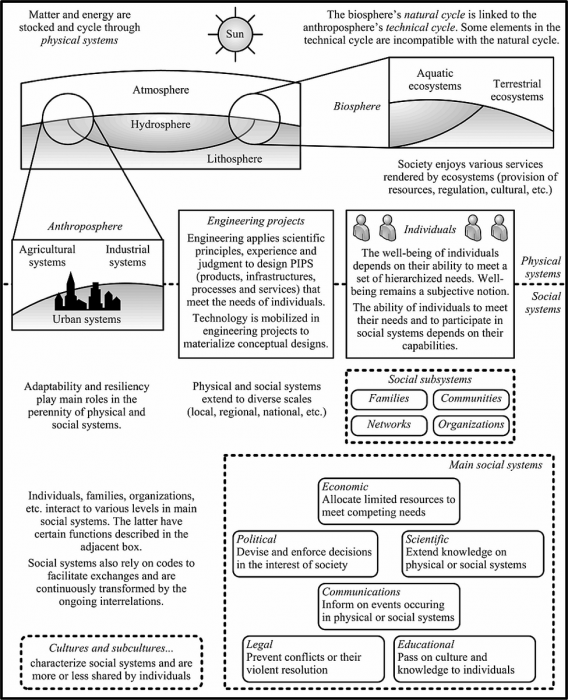
The scheme in Figure 1.20 includes both physical and social systems in consideration. Physical systems are shown by several overlapping spheres in the upper part of the diagram: the first one is biosphere (which includes the aquatic and terrestrial ecosystems), the second one is the anthroposphere (which encompasses the agricultural, industrial, and urban systems); both of these are positioned at the triple boundary between the atmosphere, lithosphere, and hydrosphere of the earth. The sun symbol above indicates the unlimited light resource. We can expand the anthroposphere box, indicating the significant role played by engineering projects as human activity. Design and engineering result in creation of products, infrastructures, processes, and services, which all increase the extent and influence of the technical cycle. The Engineering Projects box is linked to Individuals box, meaning that technical progress is incurred by and benefits individuals in the social sphere. The feedback we can assume here is possible disturbance of the natural systems due to expansion of the anthropogenic technical systems. This expansion, in turn, can jeopardize the benefits human beings and societies derive from the environment. Individuals are given a central place in the framework since physical and social systems both contribute towards their well-being. The Social Systems box at the bottom of the diagram provides a more detailed representation of those benefits, which include economic, political, scientific, legal, educational values and communications. Shown social sub-systems, such as Families, Communities, Networks, and Organizations, interact to a various degree with the main social systems and fulfill certain functions. This framework diagram is very broad, and each box could be presented as a separate sub-system with its own internal connections. But this is the big picture, which allows us to contemplate on the diversity of the factors that contribute to the sustainability of human society.
Now, think where technology fits into this diagram. Virtually, it fits anywhere at the connections of the anthropogenic spheres with the physical systems. For example, through technologies, society can utilize natural resources. We also understand that technologies can do both: reconcile the processes and matter flows between the anthroposphere and environmental spheres and create conflicts between them. Thus, engineering projects undermining the resilience or adaptability of ecosystems, social systems, or individuals might bring benefits in the short term but are likely to have long-term negative outcomes. What we call sustainable technologies are designed to render the feedbacks and connections mutually harmless or mutually beneficial in the best-case scenario. To assess technologies from this angle, we need to learn to recognize the feedbacks and effects they create on a large scale.