
Learning Objectives Self-Check
Read through the following statements/questions. You should be able to answer all of these after reading through the content on this page. After going through the content, check the boxes next to the questions/statements that you feel at least somewhat confident answering. I suggest writing or typing out your answers, but if nothing else, say them out loud to yourself. This is to help you reflect on important content, and will help you prepare for this week's quiz. It will also help lay the foundation for future course content.
Explain what it means when an appliance is 40% efficient, in terms of input and useful output.
Though many forms of energy can be converted to many others, it is important to consider how efficient the conversion process is. Energy efficiency is the percentage of "useful" energy that is converted from another form.
For example, have you ever thought about what it means to have an "efficient" light bulb, like a light emitting diode (LED)? Think about it - the purpose of using a light bulb is to provide light. Seems obvious enough, but did you know that about 90% of the energy used by an incandescent light bulb is actually converted to heat? Only about 10% is converted to light, which means that incandescents are about 10% efficient. If you are still using these old-style light bulbs, you are wasting about 90% of the money you spend on the electricity used, unless you are purposefully using them to heat your house (this is a very expensive way to heat your house, by the way). This is one reason why CFLs have become so common, and now LEDs ("light emitting diodes") - both of them are around 40% - 45% efficient, which is 4 - 4.5 times as efficient as an incandescent.
Good to Know
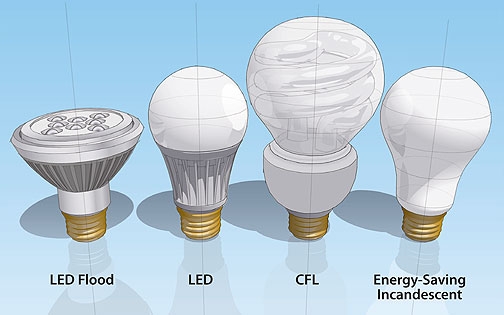
Consumers have a wide array of energy efficient lamps available to them. In addition to using electricity more efficiently, CFLs last about 10 times longer than incandescents, and LEDs around 25 times longer.
Efficiency considerations can be made for anything that uses energy. An efficient car is one that gets a lot of miles (useful "output") per gallon (energy input). An efficient home heating system, such as an electric heat pump, releases a lot of heat energy (output) for each kilowatt-hour of electric input. TVs, cell phones, airplanes, refrigerators, you name it - all have a certain efficiency. It can be used in other contexts as well. If you are efficient at work, you get a lot done (output) in a short period of time (input). In an efficient outing by a baseball or softball pitcher, not many pitches (input) were required to retire the batters (getting outs is the useful output).
This leads us to one aspect of the Second Law of Thermodynamics. A full explanation of the 2nd Law goes beyond the scope of this course, but you are welcome to watch the video below (9:29) from the Kahn Academy for a short explanation. One application of this law is that it is impossible to convert energy into a more dense, useful state without adding energy to the system. As Dr. Eric Zencey of the University of Vermont describes it, "the capacity of the energy to useful work is diminished" whenever it is transformed from one form to another (source: Is Sustainability Still Possible? p. 73). In other words, when energy is converted from one form to another, it is impossible to convert all of it. Some is "wasted" in another form, usually heat.
Let's continue with the lighting example to illustrate this. When using a light, electrical energy is converted almost entirely to light and heat (there may be a little sound energy thrown in there, but not much). Electrical energy is relatively dense, useful, and easy to control. You can store electrical energy in a battery. It is relatively easy to transport across distances without losing much. It can be used for many different things. But what about light and heat? Both of them are relatively diffuse and difficult to control. Neither is particularly useful for converting to other forms. It is very difficult to convert heat or light into another form with any kind of efficiency. Sure, you can convert heat back into electricity. In fact, this is exactly what happens in a typical power plant. But this process is very inefficient. Going further back, it is impossible to convert light, heat, or electricity back into coal (or oil, natural gas, or nuclear energy). Fossil fuels are very energy dense, and the molecules and atoms are neatly organized. Once the bonds are broken and the energy is released, there is no way to put it back together. That's the 2nd Law in action.
To Watch Now
The video below provides a very good explanation and animation of how a coal-fired power plant works. Think it's as easy as dumping a bunch of coal into a furnace and turning a turbine? Watch the video to find out. (9:28 minutes)
First Energy operates twenty power plants in the northeast United States that produce nearly seventy million megawatt-hours of electricity each year for over 4 million residential, commercial, and industrial customers. Over half of First Energy's electricity is produced by using coal compared to about 60 percent nationwide. Let's see how electricity is generated from coal by taking a virtual tour of a coal-fired power plant similar to those operated by First Energy.
This plant has three generating units that produce more than 2,000 megawatts of electricity. At full capacity, this one power plant can produce enough electricity to supply the needs of one and a half million homes and businesses. Generating electricity requires a fuel source. At this plant, the fuel source is coal which arrives mainly by a barge but also by rail and truck. Each barge delivers 1500 tons of coal - enough to keep the plant running for a couple of hours. The plant uses about 21,000 tons of coal each day, so over a million tons of coal is stockpiled next to plant. A device called a stacker reclaimer scoops coal onto a quarter mile long conveyor that can transport up to 900 tons of coal into the plant each hour.
Once inside the plant, up to a thirty-hour supply of coal can be stored in bunkers. Coal moves from the bunkers to feeders to be measured and moved to pulverizers. Each generating unit has 16 coal feeders that supply 8 pulverizers. Coal enters the pulverizer and spins in a large drum where hundreds of steel balls grind it into a fine powder.
Now a fine powder, the coal leaves the pulverizers and heads to the boiler. About 335 tons of coal can be pulverized per hour. Large fans add warm air to the powdery coal and blow it into the boiler. The boiler has miles of tubes filled with high quality water. Once inside the boiler, the coal ignites, releasing energy and generating intense heat. This changes the water inside the tubes to hot dry steam. The temperature of the steam is now about 1,000 degrees Fahrenheit. This process generates thermal energy which is transformed to mechanical energy at the turbine which is the next stop on our tour.
High pressure steam, now one thousand degrees Fahrenheit at 3500 pounds per square inch, is piped from the boiler to the first in a series of turbines. Here it expands between layers of turbine blades mounted on the turbine shaft the steam loops back to the furnace, then on to the second turbine. In this process, the steam turns the series of turbines 3600 revolutions per minute, providing power to the generator, the last in the series of machines. The generator continuously creates an electrical charge of 34481 amps at 18000 volts of electricity. From here, the electricity leaves the plant and begins its journey to customers.
After the steam has been used to create electricity, it is exhausted from the turbine and sent to a condenser to be changed back into water. Inside the condenser, steam passes over the outside of pipes filled with chilled water from the cooling towers. The steam condenses to water and returns to the boilers to repeat the steam generating process over again. As the steam condenses, the chilled water inside the condenser pipes becomes warm from the heat of the steam, so it is sent to a cooling tower. These particular towers are called natural draft cooling towers, which are designed with no moving parts. Inside, the water splashes over a series of baffles which breaks up water into small droplets. These droplets mix with air from the open bottom of the tower, evaporating some of the water and cooling the rest by as much as 27 degrees. The newly chilled water returns to the condenser to repeat the process, cooling more steam.
Since 1971, First Energy has spent more than $5 billion dollars on environmental protection. Our plants are equipped with air quality control systems to remove sulfur dioxide and particulates called fly ash. Fly ash is removed by a mechanical process, and sulfur dioxide is removed with a chemical reaction using lime. These processes take place in large ductwork scrubber trains located between a unit's boiler and its chimney. Boiler gases pass through scrubbing vessels and are sprayed with slurry, a mixture of water and lime. The sulfur dioxide particles and other pollutants are absorbed by the slurry and fall to the bottom of the vessels. A fan releases the cleansed gases through the chimney. By the time this happens, more than 99 percent of particulates have been removed, as well as ninety-two percent of sulfur dioxide. A plant similar to this one can remove over 400,000 tons of sulfur dioxide each year.
The scrubbing process creates a huge amount of a byproduct called calcium sulfite. This plant could create more than 3 million gallons of calcium sulfite slurry each day. First Energy created a process that turns that waste product into a valuable building material called gypsum which is used to make wallboard or drywall. The slurry leaving the scrubbers is thickened and pumped through a forced oxidation gypsum or fog system. Oxygen is then added changing it into gypsum which is dried treated and sent to a gypsum manufacturer to make wallboard.
First Energy recycles about a half million tons of gypsum each year - enough to manufacture wallboard for seventy thousand new homes.
Precipitators are another method of extracting coal ash, also called fly ash, which can be recycled or deposited in landfills. This mechanical process extracts fly ash left over from the combustion process. These poles, called rappers, shake fly ash from a vibrating wire. Gas from the furnace containing fly ash flows into the box. The fly ash drops into a storage chamber and is blown into silos to be loaded into a truck.
Fly ash from First Energy plants is used in manufacturing a variety of products, including concrete crowding roofing shingles, grouting, and anti skid road materials. First Energy also use a selective catalytic reduction technology or SCR to reduce nitrogen oxide emissions. SCR systems work much like a catalytic converter on a car. Flue gas containing nitrogen oxide emissions from the combustion process is mixed with ammonia. The mixed gases travel through a series of catalytic layers which causes the nitrogen oxides to react with the ammonia. The reaction converts the nitrogen oxides to water vapor and pure nitrogen, a benign chemical that makes up eighty percent of the air we breathe. Both elements are returned to the environment through the station stacks.
Now that we've taken a look at how electricity is produced and some of the environmental control protection systems used in our plants, let's take a look at how the electricity is delivered to our homes and businesses. Transformers located just outside the plant boost the power from 18,000 volts to 345,000 volts. So it can travel long distances over transmission lines to where it is needed. Substations located along the path reduce the voltage so it can be moved along distribution lines attached to the utility poles. As the electricity nears customer locations, transformers on poles step down the power for usage in homes and businesses. We hope you enjoyed seeing how electricity is produced at First Energy where our energy is working for you.
Optional - Explanation of the Second Law of Thermodynamics
The second law can be confusing, but the narrator in the video below does a pretty good job of explaining some aspects of it. Watch the Second Law of Thermodynamics (12:40 minutes) from Kahn Academy.
Let's talk about the Second Law of Thermodynamics. This law is weird. There's about 10 different ways to state it, which is one reason why it's weird. Let's start with one of the most common ways to state it, which is, if you've got a cold object and a hot object, heat will never be seen to flow spontaneously from a colder object to a hotter object. So if you have these two sitting together, maybe an ice cube and a hot piece of metal, and you make them touch, heat's going to flow between them, but we know what's gonna happen. The heat's gonna flow from the hot object to the cold object, and never the other way. At least, not spontaneously. You can force heat from a cold object to a hot object, like we do with a refrigerator or a freezer, but that's using a heat pump. And those refrigerators and freezers are doing work to force that heat from the cold region into the hot region. It won't do it spontaneously by itself. You've got to force it to do it. So what the second law says, or at least one version of it, is that that process will never be seen to happen in reverse. The heat will never be seen to flow from the cold object to the hot object. Now, you might be thinking, "Duh. "Do we really need a law to tell us that?" But it's not so obvious, because you can still conserve energy and momentum and all the other rules of physics and laws of physics by allowing heat to flow from the cold object to the hot object. In other words, let's say the cold object started with 10 Joules of thermal energy and the hot object started with ... It's hotter, so let's just say it has 30 Joules of thermal energy. You could imagine five Joules of energy going from the cold object into the hot object which would leave you with five Joules of energy for the cold object, 35 Joules of thermal energy for the hot object. You still have 40, just like you did before. You didn't break the law of conservation of energy. It's just, energy won't go that way. So why? Why is thermal energy never seen to flow from the cold object to the hot object, even though it satisfies every other known law of physics besides the second law? Well, before we answer that question, I think it'd be useful to talk about an alternate version of the second law, which looks something like this. The total disorder will never be seen to decrease. What do I mean by "disorder"? Imagine you had a room and there were blue spheres. And they're bouncing around wildly. So these all have some velocity and random directions. And when they strike a wall or each other, they lose no energy. So they keep bouncing around like crazy. And then there's another section of the room with red spheres, and these are also bouncing around randomly. They lose no energy. They keep doing their thing. Except, there's a divider in this room that doesn't allow the red spheres to go onto the blue spheres' side, and vice versa. These can't mix up. So right now, this is an ordered state because the reds are separated from the blues. So we say that this state has a certain amount of order to it. But let's imagine we removed the divider. Now what's gonna happen? Well, you'll see these things mix up. This blue sphere will move over here, and it'll bounce onto this side. This red sphere will go over here. They'll just keep getting mixed up. And at some given moment, you might find the spheres in some configuration like this. They're still bouncing around, but now they're all mixed up and we say that this state has a higher amount of disorder. This is not ordered. We say that this is more disordered, which supports the second law. The second law says, if you let things do what they wanna do spontaneously, your system will go from a more ordered state to a more disordered state. And you'll never see it go the other way. We can stand in this room and wait. But you're probably never gonna see the blue spheres line up all on the left side and the right spheres line up on the right side. With 12 total spheres, maybe if you wait long enough, a really long time, you might catch it where all the red spheres are on one side and blues are on the other. But image this. Imagine now, instead of six reds and six blues, there's 100 reds, 1,000 reds, maybe 10 to the 23d and Avogadro's number of reds, and now they're all mixed up. The odds of ever seeing them get back to this ordered state are basically zero. The probability isn't exactly zero, but the probability is very, very low that you would ever see a disordered state with that many number of particles reassemble themselves into an ordered state.
So we kind of just know that from experience and what we've seen in our day-to-day lives. But you still might be wondering, "How come? "How come we never see a disordered state Well, it basically has to do with counting. If you were to count all the possible ways of lining up the reds over here on this side and the blues on the left-hand side, there'd be a lot of combinations that would satisfy that condition. I mean, you could swap this red with that red, and this red with that red, all on the right-hand side. All these reds could get swapped around. And these blues, as well. They can get swapped around on the left-hand side. You get a large number of variations that would satisfy the condition of blues on the left, reds on the right. But now I want you to ask yourself, how many possibilities are there for having blues and reds spread out through the whole room? Well, you could probably convince yourself, there's more. And it turns out, there'll be a lot more. its position on the right-hand side somewhere. This red can get swapped out anywhere over here. I can swap a red with this blue, and this blue with this red, and this red with this red, and this blue with this blue. I can move them all over. Now that these spheres have the whole room through which they can mix, the amount of states that will have blues and reds mixed throughout the whole room will vastly outnumber the amount of states that have just reds on one side and just blues on the other side. And this simple idea is the basis for the Second Law of Thermodynamics. Roughly speaking, the Second Law of Thermodynamics holds because there are so many more disordered states than there are ordered states. Now, I'm gonna tell you something that you might not like. This particular disordered state that I have drawn, this exact one, is just as likely as this exact ordered state. In other words, if I get rid of the barrier over here, if you came in, you'd be just as likely to find the room in this exact configuration as you were to find it in this exact configuration. These two exact states are equally likely, which sounds weird. It makes you think, "Well, you're just as likely But no. This particular state is just as likely as this other particular state. But there are so many more mixed-up states than there are separated states. Even though any particular state is just as likely, since the mixed-up states vastly outnumber the separated states, if you pick one at random, it's gonna be a mixed-up state because there are so many more of them. Imagine putting these all into a hat. Imagine writing down all the possible configurations of states, ordered, disordered, in between. You put them all into a hat, you pull one out randomly, any particular state is just as likely. But since there's so many more disordered states, you pick one out randomly, it's probably gonna be mixed up. And if there's a large number of particles, you're almost certain to find it mixed up. So to help us keep these ideas straight, we need some different terms. Physicists came up with a couple terms. One is a macrostate. And a macrostate is basically saying, okay, the particle are mixed up. That's one possible macrostate. And we could be more precise. We can say, the reds and the blues can be anywhere within the box. Another possible macrostate would be to say that the particles are separated, that is to say, reds are on this side, anywhere on that side, but on the right side, and blues are on the left side, anywhere on the left side. These terms are referring to a macrostate, an overall description of what you would see. Now, there's another term, a microstate. And a microstate is a precise, exact description of the nitty-gritty details of what every particle is doing within there. If I just tell you, "The particles are mixed up," you're not gonna know exactly where they are. Similarly, if I just tell you, "They're separated," you're not gonna know exactly where they are. You'll know they'll be on the right-hand side, the red ones will, but you won't know. Maybe this red ones moves down here, maybe this red one moves up here. The microstate is an exact description. This red one's right here, going a particular speed. This blue one's right here, going a particular speed. If you specify the exact location, blue right here, blue right there, going that fast, red right here, what you're describing to me is a microstate. And so the second law, another way of thinking about it, there are more microstates for a disordered macrostate than there are micorstates for an ordered macrostate. And that's why we see systems go from order to disorder. It's really just a statistical result of counting up the possible number of states. You might be wondering, what does this have to do with heat going from hot to cold, all this talk about microstates and macrostates? Well, it's not just position that can get disordered. It's velocities that can get disordered, energy that can get disordered, and that's more of like what's happening up here. The positions of the hot molecules aren't necessarily moving over into the cold range. But the energy over here is getting dissipated into the cold area. So image it this way. Let's get rid of all this. And imagine you had a room with a gas in it, but this gas was kind of weird. At this particular moment, all the gas molecules on the right-hand side were moving really fast, and all the gas molecules on the left-hand side were moving really slow. So the room was separated into a cold region and a hot region, just like this energy is. This is ordered, or at least, somewhat ordered. It's more ordered than it's going to be. If you wait a while, this is all gonna mix up. You're gonna have some fast-moving particles over here, some slow ones over here. It's all gonna be blended together.
And so, what would you say if you were standing in here? At first, you'd feel cold because these particles don't have a lot of energy. Then you start feeling warmer and warmer. You'd say heat is flowing over to the left because you feel faster-moving particles striking your body. And so you'd rightly say that heat is moving from the right of this room to the left of this room. It flows from the hot to the cold. And that's what's happening up here. Heat flows from the hot to the cold. You might object. These are solids, I said, copper and an ice cube. A copper atom's not gonna make it over into the cold ice cube. But the energy is gonna move. So you can make the same argument over here. Don't allow these, let's say these are the copper atoms moving around fast, or at least jiggling in place rapidly. When they bump into the slower-moving water molecules in the ice cube, they're gonna give those water molecules some of their energy. And this energy's gonna become mixed up. The energy will become disordered. It will go from this ordered state, where the high energy is over here and low energy's here, to a disordered state where the energy's distributed somewhat evenly. So essentially what I'm saying is, if you consider the macrostate, where the hot molecules are separated from the cold molecules, there will be less microstates that satisfy that condition than there will be microstates that satisfy the condition for a macrostate where the energy is mixed up and you're just as likely to find a fast-moving particle on the left as you are on the right. This will have vastly more microstates, many more possible ways of making up a mixed-up state than there are microstates that create a separated state. I mean, there's gonna be a lot. I'm talking a lot of microstates that satisfy this condition for this macrostate, separated. But there will be so many more microstates for the mixed-up case, this dominates. That's why you always see heat flow from a hot object to a cold object, just because it's statistically inevitable with the large number of particles that you have here. There are so many more ways of heat flowing from hot to cold than there are from cold to hot, statistically speaking, you just never see it go the other way. Energy will always, at least spontaneously, if you let it do what it wants to, energy's always going to dissipate and evenly distribute. That's why it goes from the hot to the cold. This energy's trying to get mixed up, just because statistically, there are so many more ways for that to happen. Now, I need to tell you that there's actually a scientific term for the amount of disorder, and we call it the entropy. Physicists use the letter S to denote the entropy. And if you wanna know the formula for the entropy, you could look on Bolzmann's grave. This is Ludwig Bolzmann. He's got it on his gravestone. How awesome is that? The entropy S is k, Bolzmann's constant, times log. This is actually natural log of W. And W is the number of microstates for a particular macrostate. So you got some configuration, you wanna know the entropy? Just look at what macrostate it's in, count up how many microstates are there for that macrostate, take log of it, multiply by Bolzmann's constant, that gives you the entropy. And there's a term for this W. It's called the Multiplicity, because it's determining the multitude of microstates that satisfy the conditions for a particular macrostate. Now, entropy is cool. Entropy is weird. Entropy is somewhat mysterious and still, probably, has secrets for us to unlock here. I don't have time to go into all of them here, but if you read up on it, entropy has a role to play in the fate of the universe, the beginning of the universe, the arrow of time, maybe our perception, all kinds of facets of physics that are extremely interesting. And entropy, you always find this guy lurking around. And one place you always find entropy is in the Second Law of Thermodynamics, because it allows us a third way to state the second law, which is that the total entropy of a closed system will always be seen to increase. Technically, if it's a reversible process, the entropy could stay the same. But honestly, for all real-world processes, the entropy's gonna increase for a closed system, which is to say that the disorder increases.
Clearly, a lot of engineering goes into building a power plant. Despite the technical prowess required to convert coal into electricity, the process is extremely inefficient, as are all of the major forms of electricity generation in the U.S. and the world. Take a look at the chart below to see just how inefficient this process is for different fuels.
As you can see, as the most efficient fuel, natural gas-fired power plants are just above 40% efficient on average. Coal is closer to 30%. This, of course, means that around 70% is wasted as heat. 70%! And this does not take into consideration the losses associated with transporting the electricity across long power lines, which in the U.S. averages around 5%.
Power plants are not alone in their inefficiency. The typical internal combustion engine of a car only provides around 20% - 25% of the energy from gas to move the car. New natural gas furnaces are very efficient (95%+), but many older ones operate at lower than 80% or even 70% efficiency. This is all poor energy management in principle - it's just plain wasteful - but it is also important for a couple of other reasons, one in particular. Specifically, there is a limited amount of all of these sources, and yet they are essential for modern society. In other words, coal, oil, natural gas, and nuclear are non-renewable energy sources. (To be fair, all indications are that the world will not run out of coal, natural gas, oil, or nuclear energy terribly soon, but no one knows when it will become too expensive to use. More on that later.)
The "Fifth Fuel" (Or Perhaps the "First Fuel")
One last note before moving on to renewable and non-renewable sources. Energy efficiency is sometimes referred to as the "fifth fuel." Why do you think that is? (Hint: coal, oil, natural gas, and nuclear are the four primary fuels used globally, though that is changing as renewables are used to a greater extent.)
Increasing efficiency reduces the use of other sources of energy. Efficiency is on the demand side of energy use because it affects energy demand (think of this as how much energy is "demanded" for use.) Energy sources are the supply side of energy use because they supply the energy. By reducing demand through energy efficiency, you reduce the need for supply, which is almost like having more supply, to begin with. Hence, it is sometimes referred to as the "fifth fuel." There are tremendous opportunities for energy efficiency improvements worldwide.
Some energy efficiency advocates refer to efficiency as the "first fuel," because they feel that it should be the top priority in terms of energy management. There is some strong validity to this. Consider that a report from the American Council for an Energy Efficient Economy found that it is cheaper to reduce energy use through efficiency than it is to supply energy by any other source. Very interesting reading, if you are so inclined (and only a few pages long).
Check Your Understanding - Efficiency
Optional (But Strongly Suggested)
Now that you have completed the content, I suggest going through the Learning Objectives Self-Check list at the top of the page.