
The Smokies—and State College?
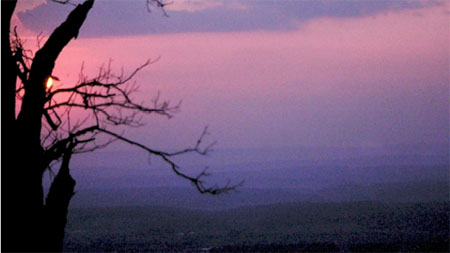
The Great Smoky Mountain National Park of North Carolina and Tennessee includes 16 mountains over 6,000 feet (about 2,000 m) high, making this generally the highest region in North America east of the Mississippi River. Gatlinburg is a mile (1.6 km) lower than Mt. Le Conte, a relief not much smaller than in many of the great mountain parks of the west, where the peaks are higher but so are the valleys. The Smokies were preserved in a park in 1926, with much of the funding for land purchases provided by J.D. Rockefeller. The Great Smokies, today, are the most-visited national park, because they combine spectacular scenery, rich biological and historical diversity, proximity to major population centers, the lure of a quick stop-off on the drive from the northeast to Florida, and a shortage of other nearby national parks to draw off the crowds. (Although we should not forget Shenandoah, connected to the Smokies by the Blue Ridge Parkway, a beautiful park in its own right.)
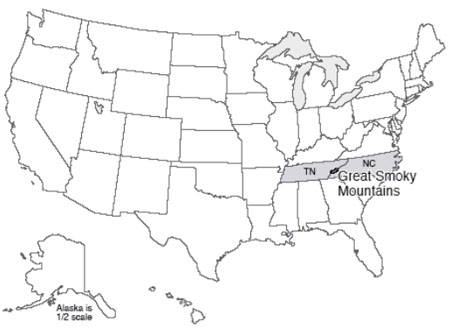
In case you’re interested, the top-ten most visited national parks in 2012 included great Smoky Mountains with 9.7 million, Grand Canyon with 4.4 million, Yosemite with 3.9 million, Yellowstone with 3.4 million, Rocky Mountain with 3.2 million, Zion with 3.0 million, Olympic with 2.8 million, Grand Teton with 2.7 million, Acadia with 2.4 million, Cuyahoga Valley with 2.3 million. Cuyahoga Valley may seem the odd-one out; it is a recent addition, seems to have been justified as a national park in part so that Ohio would have a national park, and seems to have a lot of day-picnickers from nearby Cleveland who increase the attendance a whole lot, based on Dr. Alley’s observations during a recent visit. But, Dr. Alley also believes that the Park Service is doing great things with it, and it is well worth the visit! Over 282 million people visited US national parks in 2012 (bear in mind that is with ~2 million fewer visitors than expected as a result of park closure caused by Hurricane Sandy). Note that this is nearly 90% of the whole US population. Although some people will have visited a few parks whilst others visited none, overall people are enjoying their parks!
Much interest in the Smokies centers on the historical aspects. For example, how did the early European settlers survive and flourish in this region? At Cades Cove, wonderful relicts of a bygone lifestyle are maintained in a living museum. Many visitors are also seeking to learn about the earlier Native Americans. Biologically, the Smokies host an amazing array of tree species, flowering bushes (azaleas, rhododendron, and mountain laurel in particular), wildflowers including many orchids, and more. Approximately one-third of the park is covered with "virgin" timber that was not cut by European settlers, and the regions that were logged are growing back rapidly.
Abundant rainfall and snowfall “scraped” from the sky by the high peaks feed numerous cascades and waterfalls, with trout in the pools and kingfishers by their banks. Rainfall is roughly 50 inches (1.3 m) per year in the valleys, and more than 85 inches (2 m) per year on the peaks, so the Smokies share some characteristics with temperate rainforests of the west such as in Olympic. Especially during “off-peak” times, you can get lost in the Smokies, and imagine what the Appalachians must have looked like without humans; approximately 3/4 of the park is wilderness.
Obduction Zones – The Push-Together Boundaries
The Smokies are a small part of the great Appalachian mountain chain, which extends along the coast of North America from Newfoundland through the Smokies, and then bends westward into Oklahoma. In the Great Smokies, the mountains display a truly remarkable feature—older rocks sit on top of younger rocks! (See the diagram below.) The very high peaks are composed of hard, resistant, old metamorphic rocks (which we will explain soon), of the sort that one finds deep in a mountain range. Beneath them are younger, sedimentary rocks that were deposited in shallow seaways. Between these is a surface called a thrust fault or push-together fault. Thrust faults often show evidence of sliding—scratches and polish indicating motion in one direction, crushing or breaking of rocks, etc. In some cases elsewhere in the world where deformation is still active, thrust faulting has been observed during earthquakes. In the Smokies, the older rocks have been shoved as much as 70 miles (110 km) to reach their present position on top of the younger rocks. The picture below the diagram shows two very much smaller thrust faults, with the upper rocks shoved up to the right only a few inches, but the idea is the same.
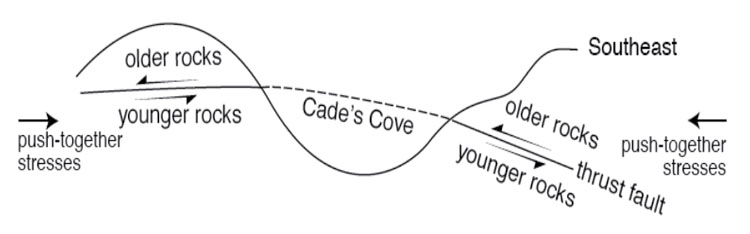
You may recall that we started with pull-apart faults at Death Valley. As shown in the diagram above, thrust faults are of the push-together type. Squeeze from either side, and one set of rocks will be pushed over another set. Each set is right-side up, but where they meet, the older rocks are on top of younger. This is seen clearly in the Great Smokies.
Farther north, in the State College, Pennsylvania area, where Drs. Alley and Anandakrishnan teach and where Dr. Alley wrote most of this text, we see a different way that rocks can respond to push-together stresses. There, in addition to some push-together thrust faults, many folds occur. Take a piece of paper, lay it on your desk, and squeeze the opposite sides towards the center. The paper will buckle into a fold. You may achieve the same effect by trying to push a carpet along the floor. Clearly, there are push-together forces involved here.
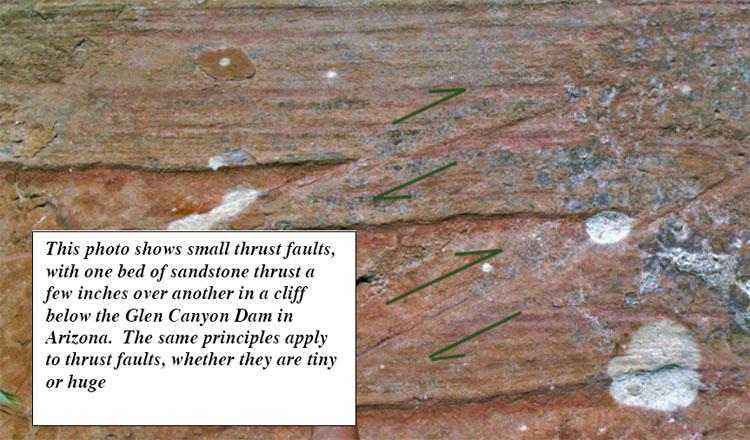
Just as pull-apart forces occur at spreading ridges, we should expect push-together forces at subduction zones, or at other collision zones. Today, the Appalachians and the east coast of South America look across the quiet sea floor of the Atlantic, across the spreading center of the mid-Atlantic ridge, to the coastlines of Africa and Europe. The coastlines on either side of the Atlantic are parallel to each other and to the mid-Atlantic ridge—slide the new and old worlds back together again, and they fit like a jigsaw puzzle. You can put all of the modern continents back together jigsaw-puzzle style. This fact, and especially the wonderful fit across the Atlantic, has figured prominently in suggesting the idea of drifting continents to scientists and other observers almost since the first decent maps were available of the Atlantic coasts. More importantly, putting the continent shapes back together jigsaw-puzzle style puts the “picture”—the geology—back together as well for events that happened while the continents were joined. For example, the tracks of a glacier run out to sea from Africa, and glacier tracks run from the sea into South America; put the continents back together, and the tracks fit together in showing the path of a single ice flow.
The oldest rocks on the Atlantic sea floor are about 150 million years old, approximately the same age as sediments that were deposited in a Death-Valley-type setting in the Newark Basin of New Jersey and elsewhere along the U.S. east coast. Evidently, the modern situation of a spreading Atlantic began about then, splitting apart a supercontinent to form the Atlantic Ocean in the same way that Baja California is being split off to open the Gulf of California.
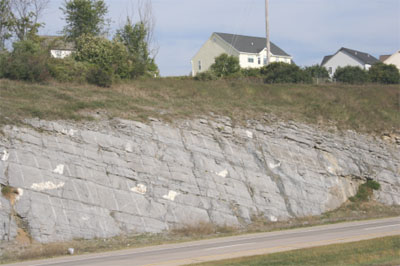
But, the Appalachian Mountains are much older than that. A story begins to emerge of a cycle—older push-together forces led to closing of a proto-Atlantic ocean that produced the Appalachians. When the proto-Atlantic was closing, subduction-zone volcanoes formed and spread ash layers across the land, much as Crater Lake/Mt. Mazama and Mt. St. Helens did more recently. (You can find some of those ash layers in many places, including the road cut along the Route 322 expressway just south of East College Avenue in the State College, PA area; see the picture at left.) Sometimes, the proto-Atlantic subduction zones formed offshore and then their volcanoes collided with the North American continent.
When the pushing stopped, the giant pile of the Appalachians, with deep, hot rocks beneath, began to fall apart in Death-Valley style, and red sandstones and mudstones accumulated in Death-Valley-style valleys along parts of the U.S. east coast. The drop in pressure as the Appalachians fell apart probably caused a convection cell in the deep mantle to rise right there, eventually forming the Atlantic Ocean.
Such a cycle, with subduction or continental collisions building mountain ranges that then spread Death-Valley style and eventually split to make ocean basins, has been played out many times over the history of the Earth. And, the fun isn’t over yet; North and South America are cruising westward toward Australia and Asia, as the Atlantic widens and the Pacific narrows. Africa is still bumping into Europe and pushing up the Alps, and India has not yet grown weary of ramming Asia to raise the Himalaya. At fingernail-growth speed, the next 100 million years or so should lead to a lot of geological high drama, but the next 100 years won’t see a whole lot of change. A good animation of this cycle can be found on YouTube.
The key to most of this is that you can sink old, cold sea floor, but you can’t sink a continent. Island arcs and continents float on the mantle too well. So, rather than going down the subduction zone with the oceanic lithosphere, the island arc or continent rides across the subduction zone for a major collision. In such a collision, called obduction, layers of rock are bent into folds such as those of the State College area, or broken into thrust faults such as those under the Blue Ridge and the Great Smokies. In the case of the Appalachians, the thrust faulting was very efficient, with older rocks sliding tens or hundreds of miles (or kilometers) over younger ones.
The Three Structural Styles
You have now seen, at least briefly, the three structural styles that are possible: pull-apart (Death Valley, spreading ridges); push-together (Crater Lake and Mt. St. Helens subduction, State College and the Great Smokies obduction); and slide-past (the San Andreas Fault in California). Pull-apart behavior involves stretching of rocks until they break, forming pull-apart or gravity faults (after being pulled apart, gravity pulls one block down past the other). Pull-apart action occurs at the spreading centers, probably where the convection cells deeper in the mantle spread apart. Push-together behavior occurs at subduction and obduction zones, and produces squeeze-together folds and faults, with the faults also known as thrust faults. Slide-past boundaries, also called transform faults, occur where two large blocks of rock move past each other but not toward or away from each other. Slide-past motion produces earthquakes without mountain ranges
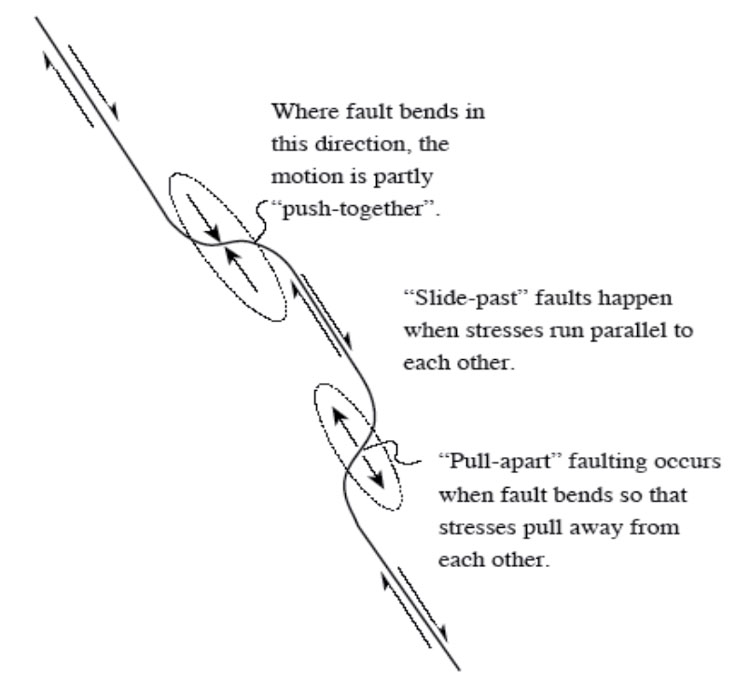
Now, you might imagine that we have oversimplified just a little. There is no law that rocks must move directly toward each other (push-together) or exactly parallel to each other (slide-past); sometimes you see an oblique motion with rocks approaching on a diagonal. Or, the rocks may pull apart on a diagonal. And a bend in a slide-past boundary may produce pull-apart or push-together features, depending on which way the bend goes relative to the motion, as shown in the diagram above. A large bend in the San Andreas Fault just north of Los Angeles gives push-together motion and some impressive mountain ranges.
Mountain ranges correspond directly to the main boundary types. Fault-block mountains—the Sierra Nevada, the Wasatch Range, the flanks of the great rift valleys of Africa, and the mid-oceanic spreading ridges—form at pull-apart margins. The mountains are high because the rocks beneath them, in the mantle, have expanded because they are the hot upwelling limbs of convection cells. Volcanic-arc mountain ranges form over subducting slabs, where some of the downgoing material melts and is erupted to form stratovolcanoes; smaller ranges (such as the Coast Ranges of the Pacific Northwest, including Olympic National Park) may form from the sediments scraped off the downgoing slab just above the trench. Continent-continent or continent/island-arc obduction collisions occur at convergent or push-together boundaries as well, producing folded and thrust-faulted mountain ranges.
Why the Great Smokies Are Still So High
Remember that crustal rocks are the low-density “scum” that floats on the denser mantle. When obduction occurs, this crustal scum is crunched—it goes from long and thin to short and thick, in the same way that the front end of a car is changed when it runs into a brick wall. Then, just like an iceberg floating in water, a mountain range is a thick block of crust floating in the mantle, with most of the thickness below and only a little bit sticking above.
With an iceberg, about 9/10 of the thickness is below the water and 1/10 above. If you could instantly cut off the 1/10 that is above water, the iceberg would pop up to almost as high as before. A 100-foot-high berg would have 10 feet above the water and 90 feet below. Cut off the top 10 feet, and it is a 90-foot berg with 9 feet, or 1/10, above the water and 81 feet below. So removing 10 feet from the top shortens the ice above water by 1 foot, and the ice below the water by 9 feet. With mountain ranges, the density contrast between crust and mantle is larger than that between ice and water—only about 6/7 of a mountain range projects down to form the root, and 1/7 projects up to form the range.
It remains, however, that if you erode a mountain range, some of the root is freed to float upward. Only by eroding the equivalent of 7 mountain ranges can you eliminate the mountain range entirely. So the Appalachians, despite having been deeply eroded, are still high because they still have a root.
The idea that things on the surface of the Earth float in soft, denser material below is called isostasy, which means “equal standing”—that each column of rocks on Earth has the same weight or standing. Lower-density columns then must be thicker to weigh as much as thinner, higher-density columns. The continents stand above the oceans because the silica-rich continental crust is lower in density than the silica-poor sea-floor crust. The mountain ranges stand above the plains because the thick, low-density roots of the mountains have displaced some of the high-density mantle that is found beneath the plains, or because the rocks beneath the mountains are especially hot and so low in density.
Put a big weight on a piece of crust (say, an ice sheet, or the Mississippi Delta, or a mountain range) and that piece of crust sinks, pushing up material around it in the same way that the surface of a water bed sinks beneath your posterior when you sit down, while the surface is pushed up around you by the water that is shoved sideways. The rising and sinking of the land are slower than for a water bed—thousands of years rather than seconds—because the hot, soft, deep mantle flows a lot slower than water does. But for a mountain range over 100 million years old, a few thousand years doesn’t mean much.
Notice something else fascinating; when a mountain range is being eroded, the top is taken off, and rocks below bob up. Those are taken off, with their place taken by more rocks from below. Pretty soon, the rocks at the surface have come from far down in the Earth, where temperatures and pressures are high. And as you might imagine, high temperatures and pressures change rocks. The rocks around State College, PA have not been “pressure-cooked” much, but the rocks around Philadelphia have been; they tell the story of a great mountain range that fell apart, leaving the remnant that we know as the Appalachians. The rocks in Rocky Mountain National Park are similar to those in Philadelphia, having been deep and now occurring at the surface. Let’s go take a quick look.