
Raising the Rockies
As noted in the text, the history of the West is quite complex, with some big questions not yet answered, and more work to be done. The most widely accepted history, with the most scientific support, is sketched in the text and given in a bit more detail just below and in the Optional Rock Video Review, The Hanging Wall.
Much evidence indicates that beginning about 100 million years ago, the subduction zone in the west grew shallower. The Pacific sea floor that was sinking under what is now Seattle is called the Farallon Plate, and rather than going down somewhat smoothly into the deeper mantle, the Farallon began to move along just beneath the lithosphere of the North American plate. The text notes that the plate was slowly getting warmer over time and thus more buoyant because the ridge and trench were getting closer together, so the plate was having less and less time to cool off before it went down the subduction zone. Another reason, and perhaps a more important reason, may be that a huge volcanic outpouring on the sea floor more than 100 million years ago made a thick layer of not-very-dense rock, which began going down the subduction zone about 100 million years ago. Where smaller bumps on the sea floor are going down subduction zones today, as off Costa Rica, features similar to those seen in the US West are forming, so the bigger features of the West are easy to explain this way.
Anyway, friction between the top of this buoyant Farallon Plate and the rocks above it—the rocks that we see in the Rocky Mountains and elsewhere in the US West—began to squeeze, bend and break those rocks above. The breaks are thrust faults, with older rocks thrust upward and over younger rocks to make the mountains. You will recall that the rocks above the fault are the “hanging wall”, which gives rise to the pun in the Rock Video.
Where a fault didn’t break all the way to the surface, it often raised the rocks above (think of lying on your back in bed under covers, and then raising your knees—the cover draping over your knees are the unbroken rocks). If the rocks were raised in a more-or-less circular pattern when viewed from above, we call the feature a dome; if shaped more like a US football when viewed from above, it may be called an arch, and a few other names are sometimes used. Such uplifts gave us the Black Hills of South Dakota, the Waterpocket Fold of Capitol Reef National Park, the Kaibab Uplift that the Grand Canyon cuts through, the beautiful San Rafael Swell of central Utah, and many other features of the West. These events mostly happened soon after 100 million years ago, during what we now call the Sevier Orogeny, and somewhat more recently in the Laramide Orogeny—we see more faults from the Sevier, and more uplifts from the Laramide.
The squeezing and thrusting of mountains caused some downwarping as well as upwarping. One of the downwarps held the lake in which the pink limestones were deposited that we now see at Bryce and Cedar Breaks, and similar lakes gave us the Green River limestones that produce such fantastic fish fossils from farther north in Utah. Oil is found in the west in rocks that accumulated in such downwarps, too.
The Farallon Plate probably eventually broke, and a new, “normal” subduction zone started up, feeding Mt. St. Helens and Mt. Rainier in the west. The Farallon Slab is now sinking beneath the eastern part of the US. As it sinks, it creates space into which hot rock flows slowly, and this may be helping “rejuvenate” the Appalachians, so the Great Smokies are a bit higher than they would be otherwise because of processes traceable back to the Rockies and the Farallon. Why the Farallon “decided” to sink eventually may be because, as it ran into thick rocks beneath the west, either some of the low-density parts were peeled off so the higher-density ones could sink, or the low-density ones were shoved deep enough that the pressure changed the mineral structures, making denser things that can sink.
Anyway, there is still much to discover about the western US. But, to catch a light-hearted version of what we know, check out the tale of a geology student trying not to be hung on the Hanging Wall.
[MUSIC PLAYING] PROFESSOR RICHARD ALLEY: (SINGING) I came out from the Eastlands, and so no-one made a slip. I showed 'em my intentions with a Brunton on my hip. I came out to where the mountains, cliffs, and mesas tower tall, to read the planet's story written on the hanging wall, on the hanging wall.
[MUSIC PLAYING]
I was hungry, young, and foolish when I started on my quest. But with a hammer, and a field book, and a map that pointed west, I left behind the ridges that the rivers rounded small to learn the truth or perish here hung from the hanging wall, from the hanging wall.
100 million years ago beneath Pacific brine, the Farallon was sinking down subducting smooth and fine 'til the slab of thickened sea floor tried to squeeze through spaces small. And the squeeze is on the westlands that would make the hanging wall, make the hanging wall. Severe Sevier orogeny, a thin-skinned thrusting belt, as the friction of the buoyant, thickened ocean plate was felt. It broke the rocks and thrust from westward raising mountains tall. Older rocks thrust over young to make the hanging wall, make the hanging wall.
[MUSIC PLAYING]
As the thickened crust moved eastward, and then turned to sink anew, the Laramide Orogeny raised domes and arches too. The Black Hills, Water Pocket, and the Kaibab as well, Wind Rivers and Uintas and the great San Rafael Swell, great San Rafael Swell.
From mountains rising in the west, a heavy load was shed, and now basal conglomerate records that river bed. But fining up and liming up soon gave us what it takes to wash the West's interior, Bryce and Green River Lakes, Bryce, Green River Lakes. But the spreading ridge, it hit the trench to make a slab window, a growing space that filled in with a hot, upwelling flow. The squeeze relaxed, the mountains spread, and though it may seem strange, new hanging walls dropped downward, normal faults, basin and range, normal faults, basin and range.
Volcanoes leaked up some new faults, but in other outcrops clear. In the footwalls of young normal faults, old thrust faults now appear. I can read the mighty story and it leaves me feeling small. I lift the map another day safe from the hanging wall, from the hanging wall.
I'm older now and wiser as I head back from my trek. But I won't die with a hempen rope around a stretched-out neck. There's still more to learn tomorrow as it holds me in it's thrall. I'll be back to read what else is written on hanging wall, on the hanging wall. Hanging wall, hanging wall, I'll be back to read what else is written on the hanging wall.
Still more about plate tectonics and related topics
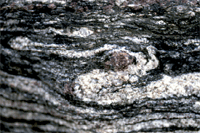
What happens when you "cook" a rock metamorphically depends on how hot, and what chemically active fluids are present, and the pressure and deviatoric stress. Both pressure and stress have units of force per area, and represent a "push" on a material. Pressure is the part of the stress that is the same in all directions--it squeezes rocks or fluids to make them smaller, but doesn't tend to change their shape. Deviatoric stress, sometimes just referred to as stress, is an extra push or pull in one or more directions, and does change the shape. Deviatoric stress has been involved in aligning the mineral grains of most metamorphic rocks into layers, or folia. Chemically active fluids—water, carbon dioxide, methane, etc.—can add or subtract chemicals, lower melting points, and dissolve and reprecipitate chemicals.
Materials that are stressed deviatorically can have one of three responses. The materials may bend and, when you release the stress, snap back (elastic deformation), they may bend permanently (plastic deformation or creep), or they may break. We have already seen examples of all of these. Earthquakes are caused by the snap-back of rocks near faults following bending. Faults such as the San Andreas are the result of breakage, and folds such as those around State College, or those in the rocks exposed in the heart of Rocky Mountain, are the result of plastic deformation.
Whether a rock bends elastically or plastically, or breaks, depends on the rock itself and on several other factors: heat (or more properly, how close a material is to melting), pressure, deviatoric stress, and even the chemically active fluids, acting over time. Elastic deformation is favored by low stresses and high pressures (to prevent breakage) and low temperatures (to prevent creep). Plastic deformation is favored by low stresses and high pressures (to prevent breakage) and high temperatures (to allow creep). Fracture is favored by high stresses and low pressures (to allow breakage), together with low temperatures (to prevent creep). Pressure matters in breakage because, to break a material, the two sides must be pulled apart, which increases the size of the material. When pressure is high, this is difficult to achieve. Fluids generally soften rocks and promote creep, although the details depend on the fluid and the rock involved. You will often see that the rocks in a region will have undergone both permanent folding and breakage, and may be bent and ready to snap back, with different modes of deformation more important in different places. Figuring this all out is fascinating, as well as useful (miners, well-drillers, and many others want to know what they'll hit in the rocks, whether the rocks are about to break, what kind of cracks the oil or gas or ores may be hiding in, and much more).
Melting and Freezing with Chemistry
If you take water and freeze it, you obtain ice that is made of the same stuff as the water. Melt the ice, and you have the water back. Melting and freezing without separating chemicals is easy to understand, but is more the exception than the rule. If you take beer and start to freeze it slowly, the crystals that form will be almost pure water ice, although each crystal typically will start to grow on a small impurity particle in the beer. Filter the crystals out, and you will have cleaned the remaining beer marginally, and you will have increased its alcohol content. Hire some advertising agents, and you have a “new” product to pitch: ice beer. (If you're under age, please substitute iced root beer. You'll end up with a lot of ice and a little sugar water.)
In the world of rocks, things are even more complex than with (root) beer. Suppose you take a piece of granite (containing quartz, mica, potassium feldspar, and a sodium-rich sodium-calcium feldspar) and melt it by heating it hot enough to melt basalt. Suppose you then start to cool it. The first minerals to crystallize may be a little bit of olivine, and a calcium-rich sodium-calcium feldspar. Cool the melt a little more, and the olivine and remaining melt react to make pyroxene, while the feldspar and melt react to make more more-sodium-rich feldspar. Keep cooling, and eventually you will get the sodium-rich feldspar and the mica, followed by the potassium feldspar and the quartz.
This ideal sequence may not be observed in many situations, but portions of it are well-known in laboratory experiments and in nature. In general, the first things to crystallize are poorer in silica, sodium, potassium and aluminum, and richer in iron, magnesium and calcium than the melt from which they grew. (Note that there are MgSiO3 pyroxenes and Mg2SiO4 olivines, or mixtures in which the Mg and Fe substitute for each other because they are almost the same size and have the same electric charge.) As the temperature drops, the early minerals react with the melt to make new minerals that are more like the original melt in composition. However, if the early minerals are removed from the melt, perhaps by settling to the bottom, they may be preserved, and exceptionally silica-rich rocks will be formed from the minerals that grow from the remaining melt. For more on the minerals, take a look at our "Sidebar" next.
Sidebar: Minerals and Rocks
If you throw a bunch of typical Earth chemicals into a pot, melt them, and cool them slowly, you will find that only certain things grow. You might, for example, find the mineral quartz (SiO2), or the mineral pyroxene (FeSiO3) or the mineral olivine (Fe2SiO4). You will not find something midway between olivine and pyroxene; it doesn’t exist. Nature puts the chemicals together in certain ways, and only certain ways. It is a little bit like building with Tinkertoys—there are only certain holes you can put the sticks into, which fix the angles at which you can build things.
Minerals are orderly—the same basic structure is repeated over and over and over (say, a silicon surrounded by four oxygens, each oxygen in contact with an iron that then contacts another oxygen that is one of four around another silicon, which is the structure of olivine). When minerals are allowed to grow freely, they assume certain shapes that look as if a gemstone-cutter had shaped them. The faces on such crystals are controlled by the underlying order of the chemicals. The classification of minerals is based on the chemical composition, and on the structure in those cases when a single composition can assume one of two or a few different structures.
Rocks are collections of minerals. One can have an all-olivine rock, or an all-pyroxene rock, or a mostly-olivine/some-pyroxene rock, or any other possible combination. We humans have chosen to classify rocks based first on their origin, and then on other characteristics such as their grain size, or their composition, or more details of their origin. The main subdivisions are igneous (rocks that formed from cooled magma or lava), sedimentary (those formed from pieces of pre-existing rocks, or from such pieces that dissolved in water and then crystallized from it), and metamorphic (those formed from igneous, sedimentary, or older metamorphic rocks by the action of heat, pressure, stress and chemically active fluids).
The classification of igneous rocks is next (we'll do igneous and then metamorphic, and save the classification of sedimentary rocks for later in the semester). We distinguish coarse-grained rocks that cooled slowly from magma deep in the Earth, and fine-grained rocks that cooled rapidly from lava at the surface; the extreme case is obsidian, a glass that cooled too rapidly to allow the high-silica types. Low-silica rocks contain the minerals olivine, pyroxene, and calcium-aluminum-rich feldspars. High-silica types include quartz, potassium- and sodium- rich/aluminum-poor feldspars, and mica. Putting these together (grain size and composition) allows us to draw the following grid:
-- | Low Silica | Medium Silica | High Silica |
---|---|---|---|
Small Grains | Basalt | Andesite | Rhyolite |
Large Grains | Gabbro | Diorite | Granite |
Of these, basalt dominates the sea floors, andesite dominates island arcs, and granite to diorite are common in the hearts of mountain ranges. Many other types occur, but these are the most important ones.
Side Bar: Metamorphic Rock Classification
The commonest sedimentary rock is shale or mud rock, and the commonest metamorphic rocks are formed from sedimentary rocks. For our purposes, then, we will just list the metamorphics that are formed from shale. With increasing heat (or time), the crystals get bigger, and some new minerals are formed. The general trend is shale (essentially mud rock), slate (harder, clinks rather than thuds when you rap it, but with grains too small to see), schist (lots of micas, grains visible to the naked eye), and gneiss (minerals have separated into dark and light layers). All of these are foliated—they appear layered. The foliations in shale come from sedimentation of small clay flakes. Those in slate, schist and gneiss come from alignment of mica grains that grow in the rock, in a direction controlled by the squeeze in the mountain range. Contact metamorphic rocks—those around igneous intrusions—don’t have the squeeze of mountain ranges and so aren’t foliated. But contact metamorphic rocks are heated, and often made wet by water that comes from the magma or by surface water that is driven to convect through spaces in the rocks by the heat of the magma. The commonest mineral in many is amphibole, a water-bearing silicate with four silicons to eleven oxygens. The commonest rock of this sort contains a lot of amphibole, and is called amphibolite.So Why Did North America Run Over the Spreading Ridge in the Pacific?
Good question. We’re not sure. But we do know that mid-ocean ridges are high because they are hot. Plates will slide off high spots, heading toward low places. So, North and South America are being pushed westward because they are sliding off the mid-Atlantic spreading ridge. If the spreading center in the Pacific simply sat still for a long while (which it probably did, more-or-less), then eventually the Americas would get to it, which they seem to be doing now.